DL-Homocysteine is a natural product found in Arabidopsis thaliana and Saccharomyces cerevisiae with data available.
Homocysteine is a uremic toxin. Uremic toxins can be subdivided into three major groups based upon their chemical and physical characteristics: 1) small, water-soluble, non-protein-bound compounds, such as urea; 2) small, lipid-soluble and/or protein-bound compounds, such as the phenols and 3) larger so-called middle-molecules, such as beta2-microglobulin. Chronic exposure of uremic toxins can lead to a number of conditions including renal damage, chronic kidney disease and cardiovascular disease. Homocysteine is a sulfur-containing amino acid that arises during methionine metabolism. Although its concentration in plasma is only about 10 micromolar (uM), even moderate hyperhomocysteinemia is associated with increased incidence of cardiovascular disease and Alzheimer's disease. Elevations in plasma homocysteine are commonly found as a result of vitamin deficiencies, polymorphisms of enzymes of methionine metabolism, and renal disease. Pyridoxal, folic acid, riboflavin, and Vitamin B(12) are all required for methionine metabolism, and deficiency of each of these vitamins result in elevated plasma homocysteine. A polymorphism of methylenetetrahydrofolate reductase (C677T), which is quite common in most populations with a homozygosity rate of 10-15 %, is associated with moderate hyperhomocysteinemia, especially in the context of marginal folate intake. Plasma homocysteine is inversely related to plasma creatinine in patients with renal disease. This is due to an impairment in homocysteine removal in renal disease. Homocysteine is an independent cardiovascular disease (CVD) risk factor modifiable by nutrition and possibly exercise. Homocysteine was first identified as an important biological compound in 1932 and linked with human disease in 1962 when elevated urinary homocysteine levels were found in children with mental retardation. This condition, called homocysteinuria, was later associated with premature occlusive CVD, even in children. These observations led to research investigating the relationship of elevated homocysteine levels and CVD in a wide variety of populations including middle age and elderly men and women with and without traditional risk factors for CVD. (A3281, A3282).
A thiol-containing amino acid formed by a demethylation of METHIONINE.
L-Homocysteine
CAS No.: 6027-13-0
Cat. No.: VC21536891
Molecular Formula: C4H9NO2S
Molecular Weight: 135.19 g/mol
* For research use only. Not for human or veterinary use.
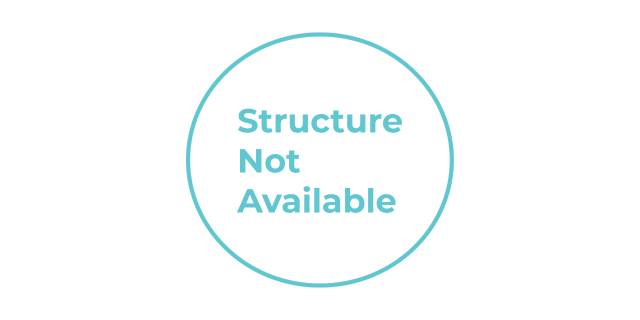
Specification
CAS No. | 6027-13-0 |
---|---|
Molecular Formula | C4H9NO2S |
Molecular Weight | 135.19 g/mol |
IUPAC Name | 2-amino-4-sulfanylbutanoic acid |
Standard InChI | InChI=1S/C4H9NO2S/c5-3(1-2-8)4(6)7/h3,8H,1-2,5H2,(H,6,7) |
Standard InChI Key | FFFHZYDWPBMWHY-UHFFFAOYSA-N |
Isomeric SMILES | C(CS)[C@@H](C(=O)O)N |
SMILES | C(CS)C(C(=O)O)N |
Canonical SMILES | C(CS)C(C(=O)O)N |
Melting Point | 232 - 233 °C |
Introduction
Discovery and Historical Background
Following its discovery, a significant milestone in homocysteine research was the first clinical descriptions of the inborn error of metabolism disorder "homocystinuria" and the subsequent discovery of severe genetic defects in enzymes involved in homocysteine metabolism as causative factors . This condition, characterized by abnormally elevated homocysteine levels in blood and urine, provided crucial insights into the pathological consequences of disrupted homocysteine metabolism. The identification of homocystinuria as a distinct clinical entity helped establish homocysteine as a biologically significant molecule with important implications for human health and disease. These early observations laid the groundwork for later investigations that would reveal the complex relationships between homocysteine metabolism and various pathological conditions.
As research progressed, scientists discovered the intricate connections between homocysteine metabolism and various B vitamins, particularly folate and vitamin B12 . The advent of convenient laboratory assays revealed mild to moderate elevation of blood homocysteine concentrations in association with vitamin insufficiencies, several diseases and disorders, some pharmaceuticals, and lifestyle factors . These discoveries fundamentally changed the understanding of homocysteine from being merely an intermediate metabolite to a clinically relevant biomarker with potential causal relationships to multiple disease states. This expanded understanding has continued to evolve, though considerable debate still exists concerning the exact contribution of elevated blood homocysteine to disease pathogenesis.
Chemical Structure and Properties
L-Homocysteine (CAS: 454-29-5) is a sulfur-containing amino acid that is structurally similar to the amino acid cysteine but with an additional methylene group in its side chain . The molecular formula of homocysteine is C4H9NO2S, with a molecular weight of approximately 135.18 g/mol. The compound contains a thiol (-SH) group, a carboxylic acid group, and an amino group, giving it both acidic and basic properties characteristic of amino acids. The presence of the thiol group is particularly significant as it allows homocysteine to participate in redox reactions and form disulfide bonds with other thiol-containing compounds, including other homocysteine molecules, forming homocystine.
The three-dimensional structure of homocysteine features a flexible carbon backbone that can adopt multiple conformations in solution, contributing to its ability to interact with various enzymes and proteins involved in its metabolism . When visualized using molecular modeling techniques, the spatial arrangement of the functional groups in homocysteine reveals potential hydrogen bonding sites and regions for nucleophilic interactions. These structural characteristics are essential for understanding how homocysteine interacts with its metabolic partners and how structural analogs might be designed for therapeutic interventions targeting homocysteine-related pathways.
Under normal physiological conditions, the concentration of homocysteine in plasma is maintained at approximately 10 μmol/L, reflecting the balance between its production and clearance . Even small deviations from this tightly regulated level can have significant biological consequences, highlighting the importance of homocysteine homeostasis in maintaining normal cellular function. The chemical properties of homocysteine, particularly its redox potential and ability to form disulfide bonds, underlie many of its biological effects and contribute to its role in both normal physiology and pathological conditions.
Metabolism and Biochemical Pathways
Methionine Metabolism and Homocysteine Formation
Homocysteine is a non-essential amino acid derived from the metabolism of dietary methionine, an essential amino acid typically consumed in excess of its requirements for protein synthesis . The conversion of methionine to homocysteine occurs through a series of enzymatic reactions collectively known as the methylation cycle. This process begins with the activation of methionine by the addition of an adenosyl group from ATP, forming S-adenosylmethionine (SAM), often referred to as the "universal methyl donor" . SAM participates in numerous methylation reactions throughout the body, transferring its methyl group to various acceptor molecules including DNA, RNA, proteins, phospholipids, and neurotransmitters. These methylation reactions are critical for gene expression, cell signaling, and numerous other cellular processes.
Following the transfer of its methyl group, SAM is converted to S-adenosylhomocysteine (SAH), which is subsequently hydrolyzed to form homocysteine and adenosine . This reaction is catalyzed by the enzyme S-adenosylhomocysteine hydrolase and represents a critical juncture in homocysteine metabolism. The equilibrium of this reaction strongly favors SAH synthesis rather than hydrolysis, but under normal physiological conditions, both adenosine and homocysteine are rapidly removed, driving the reaction toward homocysteine formation. The rate of this conversion and the resulting homocysteine levels are influenced by multiple factors, including dietary methionine intake, methylation demands, and the activity of enzymes involved in homocysteine metabolism.
The methylation cycle represents a crucial metabolic pathway that links methionine metabolism with epigenetic regulation, neurotransmitter synthesis, phospholipid production, and numerous other essential cellular processes . Disruptions in this cycle can have far-reaching consequences for cellular function and organism health. The central position of homocysteine in this pathway makes it a sensitive indicator of methylation status and a potential contributor to pathological conditions when its levels become dysregulated. Understanding this metabolic context is essential for interpreting the significance of altered homocysteine levels in various physiological and pathological states.
Regulation of Homocysteine Metabolism
The balance between homocysteine remethylation and transsulfuration is elegantly regulated by intracellular levels of S-adenosylmethionine (SAM) . SAM serves as both an allosteric activator of cystathionine β-synthase and an allosteric inhibitor of methylenetetrahydrofolate reductase (MTHFR), the enzyme that produces 5-methyltetrahydrofolate for the remethylation pathway . When cellular SAM concentrations are high, as would occur after consumption of a protein-rich meal containing methionine, SAM activates cystathionine β-synthase and inhibits MTHFR. This coordinated regulation promotes homocysteine catabolism through the transsulfuration pathway while diminishing remethylation, effectively directing excess homocysteine toward elimination rather than recycling.
Conversely, when cellular SAM concentrations are low, MTHFR activity proceeds uninhibited, increasing the production of 5-methyltetrahydrofolate for remethylation, while cystathionine β-synthase activity diminishes . This regulatory arrangement conserves homocysteine for methionine synthesis when dietary methionine is limited, ensuring that essential methylation reactions can continue even during periods of restricted protein intake. Through this mechanism, SAM functions as a metabolic "sensor" of methionine status, coordinating the activities of the remethylation and transsulfuration pathways to maintain optimal methionine availability for cellular needs.
The implications of this allosteric regulation are significant for understanding hyperhomocysteinemia. Elevation of homocysteine in the blood typically results from conditions in which the cell is no longer capable of coordinating between the remethylation and transsulfuration pathways . For example, impairment of the remethylation pathway, due to folate or vitamin B12 deficiency or genetic defects in MTHFR or methionine synthase, leads to decreased intracellular SAM concentrations. This thwarts the induction of transsulfuration and diminishes catabolism of excess homocysteine, resulting in accumulation and export of homocysteine into the bloodstream. Similarly, impairment of transsulfuration due to vitamin B6 deficiency or defects in cystathionine β-synthase leads to increased SAM concentrations, inhibition of MTHFR, and reduced remethylation, again resulting in homocysteine accumulation . This understanding explains why disturbances in either pathway can manifest as elevated blood homocysteine levels.
Genetic Determinants of Homocysteine Levels
Cystathionine β-Synthase Deficiency
The most severe form of hyperhomocysteinemia results from rare genetic disorders affecting enzymes involved in homocysteine metabolism. Among these, deficiency of cystathionine β-synthase represents the most common cause of homocystinuria, a condition characterized by extremely elevated homocysteine levels in blood and urine. This autosomal recessive disorder occurs in approximately 1 in 200,000 to 335,000 births worldwide, with higher frequencies in certain populations due to founder effects. Affected individuals typically have plasma homocysteine concentrations exceeding 100 μmol/L, far above the normal range of 5-15 μmol/L. The clinical manifestations of this disorder include developmental delays, intellectual disability, skeletal abnormalities (often resembling Marfan syndrome), osteoporosis, ocular abnormalities (especially ectopia lentis), and a markedly increased risk of thromboembolic events at an early age.
The genetic basis of cystathionine β-synthase deficiency involves mutations in the CBS gene, which encodes the enzyme responsible for initiating the transsulfuration pathway. More than 150 different mutations have been identified, with varying effects on enzyme activity and clinical presentation. Some mutations result in complete loss of enzyme function, while others permit residual activity, generally correlating with the severity of clinical symptoms. Interestingly, a subset of patients with cystathionine β-synthase deficiency respond to high-dose vitamin B6 supplementation with significant reductions in homocysteine levels, reflecting mutations that affect the binding of this cofactor rather than the catalytic function of the enzyme itself. This vitamin B6-responsive phenotype generally correlates with milder clinical manifestations and better long-term outcomes.
Methylenetetrahydrofolate Reductase Deficiency
Methylenetetrahydrofolate reductase (MTHFR) deficiency represents another important genetic cause of hyperhomocysteinemia. As described earlier, MTHFR catalyzes the conversion of 5,10-methylenetetrahydrofolate to 5-methyltetrahydrofolate, which serves as the methyl donor for homocysteine remethylation . Severe MTHFR deficiency is a rare autosomal recessive disorder characterized by developmental delay, seizures, microcephaly, and other neurological abnormalities, along with markedly elevated homocysteine levels. At least 40 rare MTHFR gene variants have been identified in individuals with decreased or absent enzyme activity . Treatment for this condition typically includes high-dose betaine supplementation, which maximizes the conversion of homocysteine to methionine via the folate- and vitamin B12-independent pathway, along with supplements of folic acid and vitamin B12 to maximize any residual MTHFR activity .
Far more common than severe MTHFR deficiency is a polymorphic variant in the MTHFR gene, specifically the 677C->T substitution, which causes an alanine to valine change in the enzyme's amino acid sequence . This variant results in a thermolabile form of MTHFR with reduced activity, leading to increased plasma homocysteine levels, particularly when folate or riboflavin status is suboptimal . Homozygosity for the 677T variant (TT genotype) occurs in approximately 10-15% of the general population, with considerable ethnic variation—lower prevalence in individuals of Black African descent and higher prevalence in Hispanic and some Asian populations . The homozygous variant is associated with increased risk of coronary artery disease, venous thrombosis, and particularly cerebral infarction and stroke . It is also associated with increased blood pressure and risk of hypertension, which has been shown to respond to riboflavin supplementation, reflecting the role of this vitamin as a cofactor for MTHFR in the form of FAD .
Other Genetic Determinants
Beyond the well-characterized deficiencies of cystathionine β-synthase and MTHFR, several other genetic variants influence homocysteine metabolism and contribute to individual variation in plasma homocysteine levels. These include polymorphisms in genes encoding methionine synthase, methionine synthase reductase, cystathionine γ-lyase, and various transporters involved in the cellular uptake of B vitamins essential for homocysteine metabolism. The cumulative effect of these genetic variants, in combination with dietary and lifestyle factors, determines an individual's homocysteine level and associated disease risks. This genetic heterogeneity partly explains the variable clinical presentation of hyperhomocysteinemia and the differential response to interventions aimed at lowering homocysteine levels. Understanding these genetic determinants provides insights into the personalized management of homocysteine-related disorders and highlights potential targets for therapeutic intervention.
Homocysteine and Neurological Disorders
Mechanisms of Neurotoxicity
Elevated homocysteine levels have been implicated in various neurological disorders, with substantial evidence indicating that homocysteine can exert direct neurotoxic effects. Experimental studies have demonstrated that homocysteine is capable of triggering neuronal damage through multiple mechanisms, including oxidative stress, DNA damage, and activation of pro-apoptotic factors . In neuroblastoma cells modified to act as neuronal models, exposure to homocysteine induced a time- and concentration-dependent reduction in cell viability compared to controls . At a concentration of 80 μM, homocysteine produced 80% cell death after 5 days of incubation, while a significant reduction of cell viability to 35% was observed after 5 days of incubation with 40 μM homocysteine . These findings highlight the potentially detrimental effects of sustained exposure to elevated homocysteine levels on neuronal survival.
Homocysteine also affects cell cycle regulation in neuronal cells, with implications for neurogenesis and neuronal repair mechanisms. Research has shown that exposure to homocysteine for 3 days increased the mRNA levels for cyclins D1, E1, and A1 by two-fold, six-fold, and five-fold, respectively, although cyclin B1 levels remained unaffected . Interestingly, these elevated cyclin levels returned to baseline after 5 days of homocysteine exposure, suggesting complex temporal dynamics in the cellular response . Additional changes in cell cycle regulators included a decrease in p21 expression after 3 days of homocysteine exposure, followed by dramatic upregulation after 5 days, along with significant upregulation of p16 and reduction of phosphorylated pRB . These alterations in cell cycle regulation may contribute to the impaired neuronal function and reduced neuroplasticity observed in conditions associated with hyperhomocysteinemia.
Electrophysiological studies provide further evidence for homocysteine's direct effects on neuronal function. When applied to the central nervous system of rats through either pressure ejection or ionophoresis, homocysteine produced a dose-dependent increase in neuronal activity in 67% of cells tested . The potency of homocysteine in this regard was comparable to that of L-glutamate, suggesting that homocysteine may act as an excitatory neurotransmitter or modulator . This excitatory action could contribute to the neurological symptoms associated with homocysteine-related disorders, potentially through mechanisms involving glutamate receptors or other excitatory pathways. The combined effects of oxidative stress, DNA damage, altered gene expression, disrupted cell cycle regulation, and excitatory neurotransmission provide multiple mechanisms through which elevated homocysteine levels could contribute to neurological dysfunction and disease.
Homocysteine and Alzheimer's Disease
The relationship between homocysteine and Alzheimer's disease has been the subject of extensive research, with growing evidence supporting a potential causal connection. Epidemiological studies have consistently demonstrated an association between elevated plasma homocysteine levels and increased risk of dementia, including Alzheimer's disease . This association remains significant even after controlling for various confounding factors, suggesting an independent contribution of homocysteine to disease pathogenesis. The exact mechanisms underlying this association are still being elucidated, but likely involve multiple pathways related to the neurotoxic effects of homocysteine described above.
Several specific mechanisms have been proposed to explain how homocysteine might contribute to Alzheimer's disease pathology. Homocysteine may promote amyloid-β peptide aggregation and deposition, a hallmark feature of Alzheimer's disease. Additionally, homocysteine can enhance tau phosphorylation, potentially contributing to the formation of neurofibrillary tangles, another characteristic pathological finding in Alzheimer's disease. The oxidative stress induced by elevated homocysteine levels likely exacerbates neuronal damage and accelerates disease progression. Furthermore, disruptions in methylation processes resulting from altered homocysteine metabolism may affect gene expression patterns relevant to neuronal function and survival in Alzheimer's disease.
Intervention studies examining the effects of homocysteine-lowering strategies on cognitive function and Alzheimer's disease progression have yielded mixed results. While some studies have shown benefits of B vitamin supplementation on cognitive decline and brain atrophy in individuals with mild cognitive impairment and elevated homocysteine levels, others have failed to demonstrate significant effects on clinical outcomes. These inconsistent findings may reflect the complexity of Alzheimer's disease pathogenesis, the timing of interventions relative to disease stage, the specific populations studied, and the particular outcomes measured. Despite these challenges, the substantial evidence linking homocysteine to Alzheimer's disease suggests that addressing elevated homocysteine levels may represent one component of a multifaceted approach to preventing or managing this devastating neurodegenerative condition.
Homocysteine and Cardiovascular Disease
Epidemiological Evidence
Numerous epidemiological studies have established a strong association between elevated homocysteine levels and increased risk of cardiovascular disease. Each 5 μmol/L increase in plasma homocysteine concentration is associated with approximately a 20% increase in the risk of coronary heart disease events . This relationship has been observed across diverse populations and appears to be independent of traditional cardiovascular risk factors such as hypertension, hyperlipidemia, diabetes, and smoking. The association is particularly robust for certain cardiovascular conditions, including stroke, peripheral arterial disease, and venous thromboembolism. The consistency and strength of these epidemiological associations have led many researchers to consider homocysteine as an independent risk factor for cardiovascular disease, although debate continues regarding whether the relationship is causal or merely correlational.
Mechanisms of Vascular Damage
The mechanisms through which homocysteine contributes to vascular damage and cardiovascular disease are multifaceted and involve several pathophysiological processes. One key mechanism is endothelial dysfunction, which represents an early and critical step in atherogenesis. Homocysteine can directly impair endothelial function by reducing nitric oxide bioavailability, increasing oxidative stress, and promoting inflammation. Research has demonstrated that homocysteine is capable of initiating an inflammatory response in vascular smooth muscle cells by stimulating C-reactive protein production . This pro-inflammatory effect may contribute to the development and progression of atherosclerotic lesions.
Homocysteine also promotes vascular smooth muscle cell proliferation and migration, key processes in the formation of atherosclerotic plaques. Additionally, it enhances platelet aggregation and activation, as well as coagulation factor activity, creating a prothrombotic state that increases the risk of thrombotic events. The oxidative stress induced by elevated homocysteine levels further contributes to vascular damage through lipid peroxidation, protein modification, and DNA damage in vascular cells. These combined effects on the vascular system provide plausible biological mechanisms for the observed associations between hyperhomocysteinemia and various cardiovascular conditions.
Measurement and Testing of Homocysteine
Analytical Methods
Reference Ranges and Interpretation
There is no universal consensus about the upper reference limits for plasma homocysteine concentrations, although the 'normal' range for healthy individuals is generally considered to be between 5 and 15 μmol/L . However, it's important to note that levels as low as 6.3 μmol/L may confer an increased risk of certain conditions, suggesting that optimal levels may be lower than the conventional normal range . The interpretation of homocysteine measurements should consider various factors that influence levels, including age, sex, genetic factors, renal function, and vitamin status. Homocysteine concentrations typically increase with age and are generally higher in males than females, partly due to hormonal influences and differences in muscle mass.
When interpreting homocysteine results, it's essential to consider the clinical context and the specific question being addressed. For cardiovascular risk assessment, even modest elevations above optimal levels may be clinically meaningful, with each 5 μmol/L increase associated with approximately a 20% increase in coronary heart disease risk . For neurological conditions such as cognitive impairment and Alzheimer's disease, the threshold for increased risk may be different, although precise cut-points remain to be established. In the context of rare genetic disorders such as homocystinuria, homocysteine levels are typically markedly elevated, often exceeding 100 μmol/L, and the diagnosis involves additional biochemical and genetic testing beyond homocysteine measurement alone.
Factors Affecting Homocysteine Levels
Lifestyle Factors
Several lifestyle factors have been associated with altered homocysteine levels, providing potential targets for intervention. Smoking is consistently associated with elevated homocysteine levels, with smokers typically having 1-2 μmol/L higher plasma homocysteine than non-smokers . This effect may be mediated through multiple mechanisms, including oxidative stress, altered B vitamin metabolism, and direct effects on enzymes involved in homocysteine metabolism. Smoking cessation generally results in modest reductions in homocysteine levels, although the time course for normalization varies among individuals and may depend on the duration and intensity of prior smoking.
Physical activity and exercise training generally have favorable effects on homocysteine levels, although acute intense exercise may transiently increase plasma homocysteine. Regular moderate exercise is associated with lower homocysteine levels in most studies, potentially through improved insulin sensitivity, reduced oxidative stress, and enhanced circulation. Obesity, particularly central adiposity, is associated with higher homocysteine levels, while weight loss typically results in modest homocysteine reductions. Sleep quality and stress levels also appear to influence homocysteine metabolism, with chronic stress and poor sleep associated with elevated homocysteine in some studies, possibly mediated through inflammatory pathways and altered neuroendocrine function.
Medical Conditions and Medications
Numerous medical conditions affect homocysteine levels, either directly through altered metabolism or indirectly through nutritional status, inflammation, or other mechanisms. Renal impairment is one of the strongest determinants of elevated homocysteine, as the kidneys play a major role in homocysteine metabolism and clearance. Even mild reductions in glomerular filtration rate can lead to significant increases in plasma homocysteine, and levels are typically markedly elevated in end-stage renal disease. Thyroid dysfunction also influences homocysteine levels, with hypothyroidism associated with elevated homocysteine and hyperthyroidism potentially lowering levels. This relationship likely reflects thyroid hormone effects on enzymes involved in homocysteine metabolism.
Diabetes mellitus, particularly type 2 diabetes, is associated with altered homocysteine metabolism, although the relationship is complex and influenced by factors including renal function, medication use, and comorbidities. Insulin resistance appears to contribute to elevated homocysteine in some individuals, while diabetes-related renal impairment may have a stronger effect in others. Inflammatory conditions such as rheumatoid arthritis are associated with elevated homocysteine levels, potentially through effects on B vitamin metabolism and direct inflammatory influences on homocysteine pathways . Malignancies can also affect homocysteine levels through multiple mechanisms, including increased metabolic demands, nutritional deficiencies, and treatment effects.
Various medications influence homocysteine levels, either as a pharmacological effect or as an adverse consequence of treatment. Methotrexate, a folate antagonist used in cancer treatment and autoimmune disorders, inhibits dihydrofolate reductase and raises homocysteine levels. This effect is typically mitigated by folate supplementation in clinical practice. Anticonvulsants, particularly phenytoin, carbamazepine, and phenobarbital, can elevate homocysteine through effects on folate metabolism. Nitrous oxide irreversibly oxidizes the cobalt atom in vitamin B12, inactivating methionine synthase and raising homocysteine levels, although this effect is generally transient with typical clinical use. Proton pump inhibitors may impair vitamin B12 absorption through gastric acid suppression, potentially leading to B12 deficiency and elevated homocysteine with long-term use . Understanding these medication effects is essential for interpreting homocysteine results in clinical practice and for identifying patients who may benefit from monitoring or preventive interventions.
Therapeutic Approaches
Vitamin Supplementation
Vitamin B6 supplementation has more modest effects on fasting homocysteine levels compared to folate and vitamin B12, but may significantly reduce post-methionine load homocysteine elevations. Doses of 10-50 mg daily are typically used in clinical practice. Riboflavin supplementation is particularly effective in lowering homocysteine in individuals with the MTHFR 677TT genotype, with doses of 1.6 mg daily or higher showing significant effects in this genetic subgroup . Combined B vitamin supplementation approaches, providing all four vitamins (folate, B12, B6, and riboflavin), target multiple aspects of homocysteine metabolism and may produce more comprehensive normalization of homocysteine levels, particularly in individuals with multiple deficiencies or genetic variants affecting homocysteine metabolism.
Beyond B vitamins, other supplements that may influence homocysteine metabolism include betaine (trimethylglycine), which directly participates in the alternative homocysteine remethylation pathway . Betaine is particularly useful in managing homocysteine levels in conditions where the primary folate- and B12-dependent remethylation pathway is compromised, such as in severe MTHFR deficiency . Typical doses range from 3-6 grams daily, divided into multiple doses. N-acetylcysteine, an acetylated form of the amino acid cysteine, may support homocysteine metabolism by providing a substrate for glutathione synthesis and reducing oxidative stress. Omega-3 fatty acids have been reported to modestly lower homocysteine in some studies, potentially through anti-inflammatory effects or influences on methylation processes, although results have been inconsistent.
Dietary Strategies
Dietary approaches to managing homocysteine levels focus on ensuring adequate intake of B vitamins and other nutrients involved in homocysteine metabolism while minimizing factors that may elevate homocysteine. Consumption of folate-rich foods, including leafy green vegetables, legumes, fruits, and fortified grains, is associated with lower homocysteine levels in observational studies. Similarly, dietary sources of vitamin B12 (primarily animal products including meat, fish, dairy, and eggs) and vitamin B6 (including poultry, fish, potatoes, and bananas) contribute to maintaining normal homocysteine metabolism. In vegetarians and vegans, particular attention to vitamin B12 intake through fortified foods or supplements is essential to prevent deficiency and consequent hyperhomocysteinemia.
The Mediterranean dietary pattern, characterized by high consumption of fruits, vegetables, legumes, whole grains, fish, and olive oil, with moderate intake of wine and limited consumption of red meat and processed foods, has been associated with lower homocysteine levels in numerous studies. This beneficial effect likely reflects the combined impact of multiple nutrients and bioactive compounds in this dietary pattern. In contrast, diets high in processed foods, refined carbohydrates, and saturated fats may promote elevated homocysteine through various mechanisms, including lower B vitamin content, increased oxidative stress, and promotion of insulin resistance.
Protein intake and composition also influence homocysteine metabolism. High protein diets, particularly those rich in methionine (abundant in animal proteins), may transiently increase homocysteine production, although this effect is typically balanced by increased catabolism in individuals with normal homocysteine metabolism. Ensuring adequate protein intake while avoiding excessive methionine load may help optimize homocysteine metabolism. Additionally, consumption of choline-rich foods (including eggs, liver, and wheat germ) and betaine-rich foods (including whole grains, spinach, and beets) supports the alternative homocysteine remethylation pathway, potentially reducing homocysteine levels, particularly when the primary folate- and B12-dependent pathway is compromised.
Management of Specific Clinical Conditions
In homocystinuria due to cystathionine β-synthase deficiency, treatment approaches depend on the specific genetic defect and response to therapy. For vitamin B6-responsive forms, high-dose pyridoxine (typically 100-500 mg daily) can substantially reduce homocysteine levels and ameliorate clinical manifestations. Vitamin B6-unresponsive cases require a more comprehensive approach, including dietary methionine restriction, cysteine supplementation, and betaine administration to promote homocysteine remethylation through the alternative pathway. These interventions, particularly when initiated early in life, can prevent or minimize the severe complications associated with untreated homocystinuria, including thromboembolic events, lens dislocation, skeletal abnormalities, and developmental delays.
For MTHFR deficiency, treatment strategies include folate, vitamin B12, and betaine supplementation to maximize homocysteine remethylation. In severe cases, 5-methyltetrahydrofolate supplementation may be more effective than folic acid, as it bypasses the defective MTHFR enzyme . For the common MTHFR 677C->T polymorphism, which affects up to 10-15% of the general population, ensuring adequate folate and riboflavin status is particularly important for minimizing the impact on homocysteine levels and associated disease risks. Riboflavin supplementation has been shown to normalize homocysteine levels and reduce blood pressure in individuals with the TT genotype, highlighting the potential for genotype-directed nutritional interventions .
In managing homocysteine levels in cardiovascular disease prevention and treatment, the focus has shifted from simply lowering homocysteine to a more comprehensive approach addressing multiple cardiovascular risk factors simultaneously. While numerous trials of B vitamin supplementation have shown effective homocysteine lowering, the impact on cardiovascular outcomes has been inconsistent, suggesting that homocysteine may be a marker rather than a causal factor in some contexts, or that interventions may need to be targeted to specific high-risk subgroups or initiated earlier in the disease process. Current guidelines generally recommend considering homocysteine measurement in individuals with premature cardiovascular disease, particularly those without traditional risk factors, and addressing elevated levels through B vitamin supplementation while simultaneously managing other modifiable risk factors.
Current Research and Future Directions
Novel Therapeutic Approaches
Research into novel therapeutic approaches for managing homocysteine-related disorders includes the development of enzymes and enzyme derivatives as homocysteine-lowering agents. For example, recombinant cystathionine β-synthase could potentially address the underlying defect in homocystinuria, while modified forms of betaine-homocysteine methyltransferase might enhance the alternative remethylation pathway in various conditions. Additionally, compounds that modulate the activity or expression of key enzymes in homocysteine metabolism, such as MTHFR activators or regulators of gene expression, represent potential therapeutic strategies currently in preclinical development.
Another promising area of research involves targeted delivery systems for B vitamins and other homocysteine-lowering compounds. These approaches aim to enhance bioavailability, increase tissue-specific uptake, and improve the efficacy of existing interventions. For example, nanoparticle-based delivery systems for folate and vitamin B12 could potentially overcome absorption or transport limitations that reduce the effectiveness of oral supplementation in some individuals. Similarly, time-released formulations might provide more consistent nutrient availability, optimizing their impact on homocysteine metabolism throughout the day.
Combination therapies targeting multiple aspects of homocysteine metabolism along with related pathways represent another frontier in therapeutic development. These approaches recognize the interconnected nature of homocysteine metabolism with other biochemical pathways, including antioxidant systems, inflammatory processes, and energy metabolism. By simultaneously addressing homocysteine levels and related pathways, such multi-targeted approaches may achieve more comprehensive health benefits than homocysteine-lowering alone, particularly in complex conditions such as cardiovascular disease and neurodegenerative disorders where multiple pathological processes contribute to disease progression.
- mass of a compound required to prepare a solution of known volume and concentration
- volume of solution required to dissolve a compound of known mass to a desired concentration
- concentration of a solution resulting from a known mass of compound in a specific volume